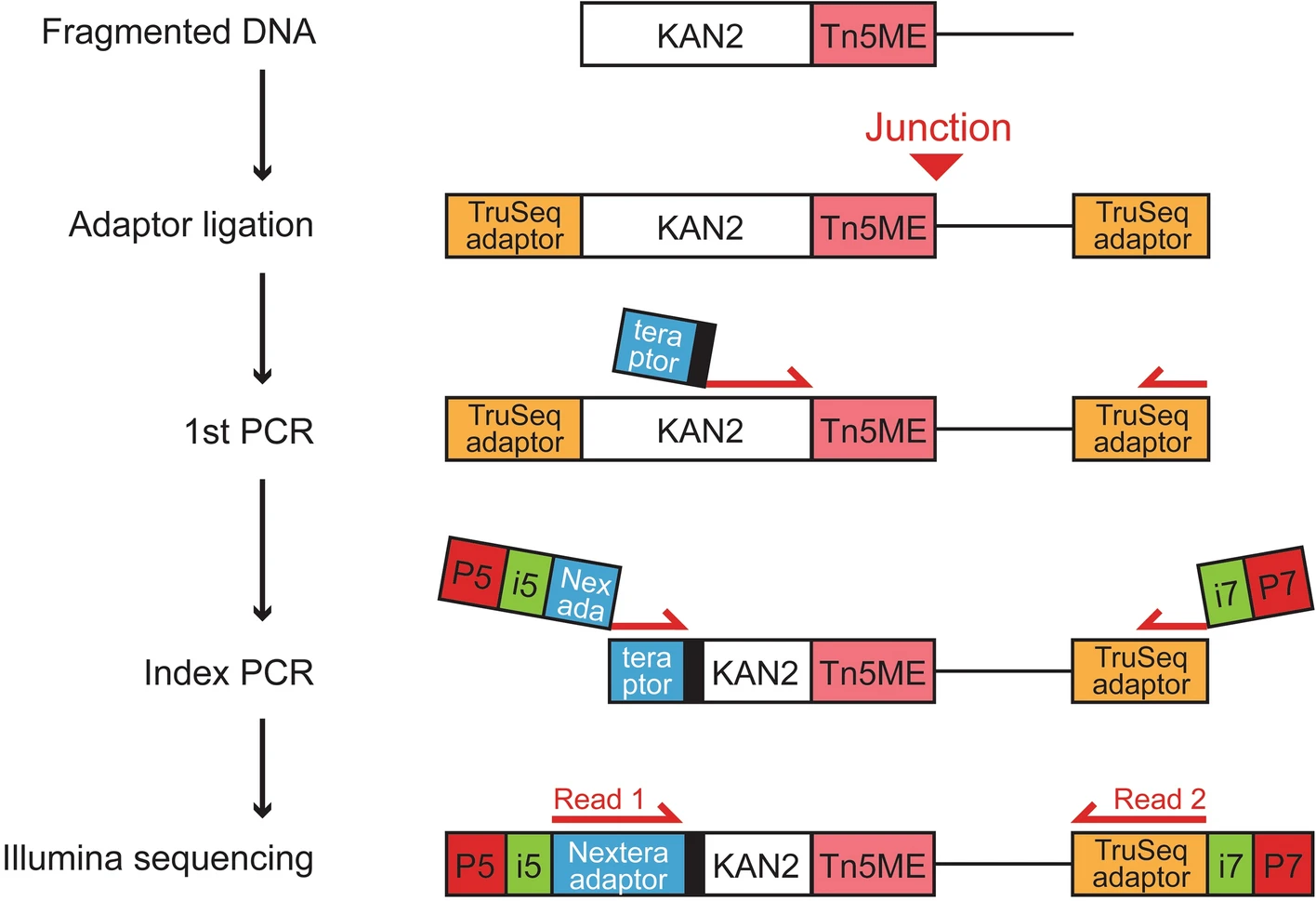
https://www.nature.com/articles/s41598-024-57537-6
- Transposon directed insertion‑site sequencing (TraDIS), a variant of transposon insertion sequencing commonly known as Tn‑Seq, is a high‑throughput assay that defines essential bacterial genes across diverse growth conditions.
- However, the variability between laboratory environments often requires laborious, time‑consuming modifications to its protocol.
- In this technical study, we aimed to refine the protocol by identifying key parameters that can impact the complexity of mutant libraries.
- Firstly, we discovered that adjusting electroporation parameters including transposome concentration, transposome assembly conditions, and cell densities can significantly improve the recovery of viable mutants for different Escherichia coli strains.
- Secondly, we found that post‑electroporation conditions, such as recovery time and the use of different mediums for selecting mutants may also impact the complexity of viable mutants in the library.
- Finally, we developed a simplified sequencing library preparation workflow based on a Nextera‑TruSeq hybrid design where ~ 80% of sequenced reads correspond to transposon‑DNA junctions.
- The technical improvements presented in our study aim to streamline TraDIS protocols, making this powerful technique more accessible for a wider scientific audience.
Introduction
- Transposon directed insertion-site sequencing (TraDIS) is a powerful high-throughput assay used to identify essential bacterial genes in various growth conditions 1 .
- This technique operates by randomly integrating Tn5 mini-transposons into the bacterial genome through the action of the transposase enzyme, a process that disrupts or alters gene function.
- While widely applied, TraDIS protocols are not standardized and often require custom modifications to suit the unique circumstances of different laboratories, such as variations in available equipment and bacterial strains.
-
These adjustments can be particularly time-consuming and labor-intensive for those new to the technique.
-
Our current study aims to streamline the construction of complex mutant libraries by identifying key parameters that enhance labor efficiency and practicality.
- We employed Escherichia coli strains from diverse sources, including freshwater environments, the human gut, and laboratory settings, to demonstrate the versatility and applicability of our methods.
- Our investigation included optimizing electroporation conditions to improve transposon insertion and mutant recovery.
- Specifically, we fine-tuned the concentration of the transposome, evaluated the effect of varying transposon DNA quantities during transposome assembly, and explored how cell density affects the outcomes of electroporation reactions.
- Following the optimization of electroporation conditions, our study investigated the impact of post-electroporation parameters on the diversity of mutants with unique insertion sites.
- We assessed the length of recovery time after electroporation and compared the efficacy of different mediums for selecting mutants, namely agar plates versus liquid broth.
- While using liquid broth is a simpler approach, it potentially compromises the complexity of the recovered mutant library, as mutants compete for shared resources before the culture can be collected.
-
These comparisons aimed to determine the most effective method for constructing complex mutant libraries while considering factors such as labor efficiency and practicality.
-
Another important aspect of TraDIS assay is a robust PCR protocol to enrich transposon-DNA junctions, while minimizing amplification of non-specific fragments without such junctions.
- Prior strategies include the use of splinkerette adaptors followed by a sequencing run with custom primers and operation protocols2,3.
- However, this strategy requires a dedicated run for TraDIS libraries, which can limit the use of a higher capacity, more cost-effective sequencer like NovaSeq.
- Another strategy is a nested PCR approach using ‘all-in-one’ primers that combine both the Illumina flow cell and transposon-specific sequences4,5.
- Although this method is effective, it involves the use of long primers (exceeding 60 base pairs), which often necessitates more expensive synthesis.
- Also, if one were to use a different transposon for the assay, this requires the synthesis of a new set of ‘all-in-one’ primers.
- To this end, we present a PCR strategy that simplifies the library preparation workflow.
- Our library construction scheme is compatible with standard TruSeq/Nextera indexing primers used for other Illumina assays, thereby reducing the overall cost.
Methods
Bacterial strains (Supplemental Table 1)
- We utilized three strains of Escherichia coli, each selected for its unique origin and characteristics.
- AB_00116 was isolated from a freshwater environment and provided by Aaron Best’s laboratory at Hope College, Holland, Michigan, USA.
- MS 69-1 was obtained from BEI Resources (HM347) and originally isolated from the colon of a control patient with abnormal histology in New York, New York, USA.
- D43HC1 was derived from a laboratory E. coli strain ATCC25922, previously characterized as multi-drug resistant after being exposed to a gut relevant-
concentration of the antipsychotic drug quetiapine in vitro for 6 weeks6.
- These strains were chosen to represent a diverse range of environmental and physiological conditions, thereby ensuring the robustness and applicability of our experimental findings across different bacterial backgrounds.
Synthesis of transposon encoding the kanamycin resistance gene (KAN2)
- Using the Twist Bioscience Gene Fragments service, we ordered a custom DNA fragment corresponding to the KAN2 transposon (1221 bp), whose full sequence is available on the product manual for the EZ-Tn5 < KAN-2 > Tnp Transposome Kit (Lucigen #TSM99K2).
- We PCR amplified 1 ng of the fragment with Q5® High-Fidelity 2X Master Mix (NEB #M0492) using a PCR primer set: Fwd 5′—/5′Phos/CTGTCTCTTATACAC
ATCTCAACCATCATCGA—3′ and Rev 5′—/5′Phos/CTGTCTCTTATACACATCTCAACCCTGAAGCT—3′ and the following PCR cycle parameters: 98 °C for 30 s, 18 cycles of (98 °C for 10 s, 69 °C for 30 s, 72 °C for 45 s), and 72 °C for 2 min (50 uL reactions in triplicate).
- After confirming the amplicon size by gel electrophoresis, we purified the amplicon using the DNA Clean & Concentrator-5 kit (Zymo Research #D4013).
- To increase the DNA concentration in the final eluate, we used three purification columns (i.e. one column for each PCR reaction).
- At the elution step, we eluted the first column with 25 uL of the fresh elution buffer for the DNA Clean & Concentrator-5 kit (10 mM Tris-HCol, ph 8.5, 0.1 mM EDTA).
- For the second and third columns, we used the first and second eluates as the elution medium.
Transposome assembly
- We purchased the unloaded Tagmentase (Tn5 transposase) from Diagenode (#C01070010-10; 2 mg/mL = 37.52 uM), and adjusted the protein concentration to 1 uM by diluting it in the storage buffer described in the manual for the EZ-Tn5 Transposase (LGC Biosearch Technologies) (50 mM Tris–HCl (pH 7.5), 100 mM NaCl, 0.1 mM EDTA, 0.1% Triton® X-100 (Rohm & Haas) and 1 mM DTT).
- To assemble the transposome, we mixed 1 part of the KAN2 transposon DNA solution, 2 parts of the diluted Tn5 at 1 uM, and 1 part of 100% glycerol, and incubated in a thermocycler at 23 °C for 60 min before use (or stored at − 20 °C if not used immediately).
Electroporation experimental setup (Basic workflow)
- To make E. coli electrocompetent, we first inoculated a colony of bacteria in 3 mL of LB medium and shake-incubated at 37 °C overnight.
- Next day, we transferred the overnight culture in fresh LB medium (1/100 dilution) and shake-incubated again at 37 °C until the optical density at 600 nm ( OD600) of the culture reached ~ 0.4.
- Cells were harvested by centrifugation at 4000g for 10 min at 4 °C, washed twice in 2/3 culture volume of sterile water (4 °C) (centrifugation after each wash), and then resuspended in 1/100 culture volume of sterile water (4 °C) (e.g. for 45 mL of culture, 30 mL water was used for each wash, and 450 uL water was used for final resuspension).
- Cells and electroporation cuvettes (1 mm gap) were kept on ice until electroporation.
- We used BioRad Gene Pulser to electroporate the bacteria using the following parameters: 2000 V, 25 uF and 200 Ω.
- Immediately after electroporation, we added 1 mL of SOC medium, pipette up and down several times, and transferred to a sterile 2 mL screw-cap tube for recovery in a shaker incubator at 37 °C (with tubes placed in a horizontal position for better aeration).
- We recorded the time constant for each electroporation reaction.
抗卡那霉素基因 (KAN2) 转座子的合成
- 我们使用 Twist Bioscience Gene Fragments 服务订购了一个自定义 DNA 片段,该片段对应于 KAN2 转座子(1221 bp),其完整序列可以在 EZ-Tn5 < KAN-2 > Tnp Transposome Kit(Lucigen #TSM99K2)的产品手册中找到。
- 我们使用 Q5® 高保真 2X Master Mix(NEB #M0492)和一组 PCR 引物:Fwd 5′—/5′Phos/CTGTCTCTTATACACATCTCAACCATCATCGA—3′ 和 Rev 5′—/5′Phos/CTGTCTCTTATACACATCTCAACCCTGAAGCT—3′,对片段进行了 PCR 扩增,使用的 PCR 循环参数为:98 °C 30秒,18个循环(98 °C 10秒,69 °C 30秒,72 °C 45秒),以及 72 °C 2分钟(50 uL 反应,三次重复)。
- 通过凝胶电泳确认了扩增产物的大小后,我们使用 DNA Clean & Concentrator-5 kit(Zymo Research #D4013)纯化了扩增产物。
- 为了增加最终洗脱液中的 DNA 浓度,我们使用了三个纯化柱(即每个 PCR 反应一个柱)。
- 在洗脱步骤中,我们用 25 uL 新鲜的洗脱缓冲液为第一个柱洗脱,该洗脱缓冲液来自于 DNA Clean & Concentrator-5 kit(10 mM Tris-HCl,pH 8.5,0.1 mM EDTA)。
- 对于第二和第三个柱,我们使用了第一和第二次洗脱液作为洗脱介质。
Transposome 组装: Tn5 transposase [转座酶] (1. protein) + KAN2 transposon DNA (2. DNA) = Tn5 transposome [转座体] (3. protein-DNA complex)
- 我们从 Diagenode 购买了未加载的 Tagmentase (Tn5 转座酶)(#C01070010-10; 2 mg/mL = 37.52 uM),并按照 EZ-Tn5 转座酶(LGC Biosearch Technologies)手册中描述的储存缓冲液(50 mM Tris–HCl (pH 7.5),100 mM NaCl,0.1 mM EDTA,0.1% Triton® X-100 (Rohm & Haas) 和 1 mM DTT)调整蛋白浓度至 1 uM。
- 为了组装 transposome,我们将 1份 KAN2 转座子 DNA 溶液、2份稀释至 1 uM 的 Tn5 以及 1份 100% 甘油混合,并在热循环仪中于 23 °C 孵育 60分钟,使用前取出(或若暂时不使用则储存于 −20 °C)。
- 转座体组装: 在这个步骤中,未装载的Tagmentase(Tn5转座酶)与转座子DNA(如KAN2转座子)按照一定比例混合,并加入甘油进行保护,然后在特定温度下孵育一段时间。这个过程的目的是使转座酶和DNA片段结合,形成可活性的转座体复合物。这种复合物可以将DNA片段插入到目标细胞的基因组中。
- 转座酶和DNA片段结合后形成的转座体(transposome)的大小主要取决于使用的DNA片段的长度。在实验中通常使用的转座子DNA可以是几百到几千碱基对长。因此,转座体的大小会略大于所使用的DNA片段的长度,加上转座酶本身的尺寸。例如,如果使用的转座子DNA片段长度为2000碱基对(大约等于2千个核苷酸),转座酶(如Tn5转座酶)则具有一定的三维结构和体积,可能将整个复合物的长度增加数十到数百纳米。转座酶一般会与DNA的特定位点结合,形成稳定的蛋白-DNA复合体。这种复合物的实际尺寸和结构取决于具体的转座酶和DNA的结合方式,以及转座酶自身的空间结构。
电转化实验设置(基础工作流程)+ Cells [4]
- 为了使大肠杆菌电转化成分子,我们首先在 3 mL LB 培养基中接种一个细菌菌落,并在 37 °C 下振荡培养过夜。
- 第二天,我们将过夜培养物转移到新鲜的 LB 培养基中(1/100 稀释)并再次在 37 °C 下振荡培养,直到培养液的光密度在 600 nm(OD600)达到约 0.4。
- 细胞通过在 4 °C 下 4000g 离心 10 分钟收集,洗涤两次,每次使用 2/3 培养体积的无菌水(4 °C)(每次洗涤后离心),然后用 1/100 培养体积的无菌水(4 °C)重新悬浮(例如,对于 45 mL 的培养物,每次洗涤使用 30 mL 水,最终重悬时使用 450 μL 水)。
- 在进行电转化前,将细胞和电转化池(1 mm 间隙)保持在冰上。
- 我们使用 BioRad Gene Pulser 进行电转化,参数为 2000 V、25 μF 和 200 Ω。
- 电转化后立即加入 1 mL SOC 培养基,上下吸吮数次,然后转移到一个无菌的 2 mL 螺旋盖试管中,在 37 °C 的摇床孵育器中恢复,试管置于水平位置以便更好地通气。
- 我们记录了每次电转化反应的时间常数。
- 尽管转座体已经组装好,但它们仍需被有效地送入宿主细胞(如大肠杆菌)内才能发挥作用。电转化是一个高效的细胞转化技术,通过对细胞暂时施加一个高电压电场,造成细胞膜上微小的孔隙,使得转座体可以通过这些孔隙进入细胞内部。在电转化后,这些孔隙会迅速自我修复,从而将外源DNA锁定在细胞内部。
Analyzing the effect of selection medium (agar plate vs. liquid culture) on mutant library complexity
- After 1 h of recovery from electroporation, we selected mutants as follows: Agar plates: We spread one portion of the culture on LB agar plates containing 40 μg/mL kanamycin (each plate received an appropriate volume of culture to generate 1000–2000 CFUs), and incubated at 37 °C overnight.
- On the next day, we first measured CFUs using a ProtoCOL3 automatic colony counter (Synbiosis), and then scraping off colonies from agar plates using a sterile cell spreader and 1 mL of LB.
- We recovered an equal number of mutants for each strain under investigation.
- We extracted DNA from a dilute sample of the pooled culture using the DNeasy Blood & Tissue kit (Qiagen) according to the manufacturer’s instructions. Liquid culture: We transferred the equal volume of the
Analyzing the effect of recovery time on mutant library complexity
- After electroporation and addition of SOC medium for each strain, we combined multiple reactions into one to normalize the potential difference in electroporation efficiency of each reaction, and then immediately split them into equal portions among 2 mL screw-cap tubes.
- All portions were shake-incubated in parallel at 37 °C, and they were spread onto LB-kanamycin plates at designated time points.
- All plates were incubated at 37 °C overnight.
- Each experiment was performed in duplicate.
- On the next day, we first measured CFUs using the ProtoCOL3 automatic colony counter, and then scraped off colonies from agar plates using a sterile cell spreader and 1 mL of LB.
- We extracted DNA from a dilute solution of the pooled culture using the DNeasy Blood & Tissue kit (Qiagen) according to the manufacturer’s instructions.
Statistics
- Statistical analysis involved applying a two-way analysis of variance (ANOVA) to assess significance, followed by Tukey’s Honest Significant Difference test for post hoc comparisons.
Construction of DNA libraries and sequencing
- We sonicated extracted DNA samples using the Covaris S2 sonicator (parameters: Intensity – 5; Duty Factor – 10; time – 90 s) to fragment the genome into 300–400 bp pieces.
- Proper fragmentation was verified via gel electrophoresis.
- We used the NEBNext Ultra II DNA Library Prep Kit for Illumina (NEB #E7103) to constructed DNA library (input = 500 ng) according to the manufacturer’s protocol (version 7.0_9/22) with the following adjustments:
- (1) During fragment size selection, we used 22.5 μL (Step 3A.2) and 10 μL (Step 3A.6) of the NEBNext Sample Purification Beads;
- (2) At “Step 4.1 PCR Amplification” we enriched the transposon-DNA junction using the following PCR parameters: 1 cycle of 98°C for 30 s, 22 cycles of [98 °C for 10 s, 65 °C for 75 s], and 1 cycle of 65 °C for 5 min (primer sequences are listed in Supplemental Table 2; and
- (3) We eluted the resulting PCR products using 52.5 uL of 10 mM Tris (pH 8.0) (Step 5.9) and collected 50 uL of the eluate in Step 5.11.
- We measured the concentration of each eluate using Qubit reagent (Invitrogen #Q32851) and adjusted concentrations of each sample to 4 ng/uL with 10 mM Tris (pH 8.0).
- Using 20 ng of 1st PCR product as input material, we conducted index PCR (50 uL reaction) with 2X KAPA HiFi HotStart ReadyMix (Roche Diagnostics #07958927001), Illumina Nextera/TruSeq P5 and TruSeq P7 index primers, and the following PCR parameters: 1 cycle of 95 °C for 3 min, 8 cycles of [95 °C for 10 s, 55 °C for 30 s, 72 °C for 30 s], and 1 cycle of 72 °C for 5 min.
- We purified the resulting product with the HighPrep PCR beads (MagBio Genomics #AC-60005) according to the manufacturer’s protocol (version v2.0) using the beads-to-sample ratio of 1:1.12.
- We pooled libraries in equimolar concentration and sequenced on the Illumina NovaSeq 6000 platform (150 bp PE).
Sequencing data analysis (TODO: NEXT_WEEK_REPERT_THE_PIPELINE_ON_THE_PAPER_DATA_and_ON_OWN_DATA!)
- We first subjected fastq reads to two rounds of filtering using the cutadapt software (ver. 4.1) to remove reads that did not have expected fragment layout.
- For the first round of filtering, we trimmed a total of 29 bp sequences from Read 1 (5′—GCA TGCAAGCTTCAGGGTTGAGATGTGTA—3′), which corresponded to the PCR anchor (20 bp) and the 5′ portion of the 19-bp Tn5 mosaic end (ME) sequence (9 bp), with a maximum of 10% mismatches allowed.
- For the second round, we trimmed the remaining 10-bp ME sequence (5′—TAAGAGACAG—3′), with no mismatch allowed.
- In both rounds of filtering, we discarded reads that did not pass the filtering criteria (‘–discard-untrimmed’), and corresponding read mates were discarded from Read 2 fastq files.
- We mapped the filtered reads (Read 1 only) to respective reference FASTA in both forward and reverse complement orientations using the bwa software (version 0.7.17-r1188) with default options.
- We defined the transposon-DNA junctions, which is equivalent to the number of unique mutants in the library, as the leftmost coordinate of the mapped reads, and we used a custom bash script to determine this.
- We first sorted the mapped reads based on their genomic coordinates using the samtools software (version 1.14) and filtered for reads that align to forward orientation (FLAG = 0 in the second column).
- We then de-duplicated reads with identical coordinates (chromosome and the leftmost position of the mapped reads) and counted the number of unique reads as ‘putative junctions’.
- We filtered out putative junctions with low number of reads (< 1 count per million reads mapped), and then classified the remaining junctions as ‘true junctions’, which are reported in the Results section.
- The code used for this analysis can be found here: https://github.com/StephanieAFlowers/TraDIS_techical_paper.git.
#### Calculate the number of putative junctions
grep -v "@PG" ${SORTED.SAM} |grep -v "@SQ" |grep -v "@HD" |awk '{if ($2 == 0) print $3"_"$4}' |uniq |wc -l
#### Calculate the number of putative junctions with low read counts (CPM < 1) to be subtracted ####
grep -v "@PG" ${SORTED.SAM} |grep -v "@SQ" |grep -v "@HD" |awk '{if ($2 == 0) print $3"_"$4}' |uniq -c |awk '{print $1}' |sort -k1,1n |uniq -c |head -${LOW_CPM_THRESHOLD} |awk 'BEGIN {OFS="\t"} {print $2,$1}' |awk '{sum += $2} END {print sum}'
Results
Diluting transposome [3] improves electroporation efficiency (稀释转座酶复合体可以提高电穿孔效率, https://zhuanlan.zhihu.com/p/664630787)
- We examined how electroporation efficiency is affected by the amount of transposome in electroporation reaction (Fig. 1A).
- To analyze this, we serially diluted the most concentrated transposome (1 U) to 0.5, 0.25, 0.1, 0.05, 0.025, and 0.01 U, mixed into electrocompetent cells, and assessed the electroporation efficiency by measuring CFUs the next day.
- All conditions for each strain were performed in duplicate. We found that CFUs increased as we used more diluted solutions of transposome, peaked around 0.05 U for AB_00116 strain or 0.1 U for MS 69–1 strain, and then declined.
- The effect of transposome concentration on electroporation efficiency, assessed via two-way ANOVA, was significant (p = 3.95e–08).
- The time constant (τ) is measured by the product of resistance and capacitance and often correlates with electroporation efficiency.
- We found that the time constants for reactions containing 1, 0.5, and 0.25 U transposome were 8.7%, 4.3%, and 2.2% lower compared to those containing less than 0.25 U, which correlated with lower CFUs (Fig. 1B).
Higher quantity of transposon DNA [2] during transposome assembly improves electroporation efficiency
- We also examined how electroporation efficiency is affected by the quantity of transposon DNA during transposome assembly (Fig. 2). To analyze this, we assembled transposomes by mixing 1 U of Tn5 with 400, 200, or 100 ng of KAN2 transposons.
- We mixed 0.05 U of the assembled transposomes with electrocompetent cells, and assessed the electroporation efficiency by counting the resulting CFUs the next day.
- All conditions were performed in duplicate.
- The CFUs were the highest for those electroporated with the transposome with 400 ng KAN2 transposon, followed by 200 ng (18.0% CFU on average relative to 400 ng), and then 100 ng, which yielded virtually no colonies like the mock-electroporated negative control.
- We assessed the effect of transposon DNA quantity on electroporation efficiency by two-way ANOVA (p = 7.95e–12).
- The time constant (τ) was not affected by the transposon quantity.
Cell density [4] in electroporation reaction affects its efficiency
- We determined how electroporation efficiency is affected by the cell density in the reaction mixture (Fig. 3).
- We mixed 0.05 U of the transposomes with cell suspension of different densities (50X, 100X or 200X of the starter culture volume), and assessed the efficiency by counting the resulting CFUs the next day.
- All conditions were performed in duplicate.
- We found that overall, 100X concentrate of the starter culture volume had the highest electroporation efficiency, followed by 50X (21.4% less efficient on average) and 200X (56.8% less efficient on average; p = 0.0014).
- The density producing the highest electroporation efficiency was somewhat strain specific.
- The time constant (τ) was not affected by cell density.
Increasing recovery time after electroporation does not significantly affect the number of unique insertion events
- Electroporation of transposome into bacterial cells is immediately followed by a recovery period in rich growth medium such as SOC.
- This recovery period provides time for bacterial cells that successfully took up the transposome to express selection marker genes.
- However, the length of recovery time is not standardized in the TraDIS assay and varies among previous studies (from 1–2 h 4,5,7,8).
- This is an important parameter to consider when creating the mutant strain library, as the number of unique mutants is often estimated from CFUs (Colony-forming unit).
- Therefore, we sought to examine the relationship between the number of CFUs and unique mutants, as a function of recovery time length.
- We hypothesized that longer recovery time would result in a lower proportion of unique mutants because longer recovery time would allow expansion of clones in culture.
- To test the hypothesis, we electroporated our E. coli cohort with the Tn5 transposome carrying the KAN2 gene, and let them recover for 0.5, 1, and 2 h before plating on kanamycin selection plates.
- After an overnight incubation (Digital bacterial incubator), we harvested the same number of colonies for each time point per strain, and determined the number of unique mutants via sequencing.
- Not surprisingly, a longer recovery time yielded higher CFUs, doubling roughly every 0.5 h of recovery time (Fig. 4A).
- Contrary to our expectation, however, we did not observe a corresponding decrease in the number of unique insertion events (p = 0.4), and the number of unique mutants recovered was somewhat strain dependent (Fig. 4B).
- For two of the three test strains (MS 69-1 and D43HC1), we found that the number of unique mutants detected were comparable across time points; whereas, for strain AB_00116, we observed a reduced number of mutants as the recovery time lengthens.
Based on the information you provided, your understanding that each colony contains an isolate with multiple insertion events is not necessarily correct. Here's a more precise breakdown:
- Transposome and Insertion Events: When transposomes are electroporated into bacterial cells, they can integrate into the genome at multiple locations. However, each individual transposome typically only carries one or a few copies of a DNA fragment, which could insert into different sites within the bacterial genome. The number of insertion events per cell depends on how many transposomes enter the cell and successfully integrate into the genome.
- Colony Formation: After electroporation, cells are allowed to recover and then plated on a selective medium that only allows the growth of cells where the transposome has successfully integrated (because it carries an antibiotic resistance gene like the KAN2 gene). Each colony that forms on the plate theoretically originates from a single cell that took up the transposome. Therefore, each colony represents a unique isolate, which could potentially have multiple insertion events if multiple transposomes entered that particular cell and integrated at different sites.
- Unique Insertion Events: The research you described was testing whether the length of recovery time after electroporation affects the number of unique insertion events within these colonies. The results indicated that the number of unique insertion events did not significantly change with different recovery times, although the number of colonies (CFUs) increased with longer recovery times.
- Conclusion: Each colony on the selective plate indeed originates from a single cell that has taken up the transposome. While it's possible for multiple insertion events to occur within a single cell (leading to possibly multiple insertion sites within the genome of the cell that founded the colony), each colony primarily represents a unique transformation event. The total number of unique insertion events across the population might not necessarily increase with longer recovery times, indicating that the dynamics of how transposomes integrate are complex and may be influenced by factors other than just the length of recovery time.
- Therefore, each colony generally represents a unique transformant, potentially with multiple insertion events.
- Combination of Sites: The diversity also involves the combination of sites where insertions occur within a single cell or across a population of cells. For example, in experiments involving transposons, some cells may have insertions at unique single sites, while others may have multiple insertions at different sites. The range and pattern of these combinations add to the diversity of insertion sites.
If it means that after long time, more colonies have the same combination of sites, or means that after long time, more different combinitions of sites occours based on the infomation: after long time, we have more colonies, but total unique insertion sites are not changed?
Based on the information provided in your earlier message about the experimental findings, it seems that extending the recovery time after electroporation results in a higher number of colonies (CFUs), but does not significantly alter the total number of unique insertion sites across all colonies. This indicates a couple of key points regarding the diversity and combination of insertion sites:
* Increase in Colonies but Steady Number of Unique Insertion Sites: As recovery time increases, more cells survive and form colonies. However, the overall diversity of insertion sites—the different combinations and locations where the transposome integrates into the genome—does not significantly change. This suggests that while there are more colonies, they may not necessarily exhibit a greater variety of insertion site combinations.
* Implications for Insertion Site Combinations: The fact that the total number of unique insertion sites remains roughly constant despite more colonies forming can suggest two things:
- Redundancy in Insertion Events: Longer recovery times might allow for more cells to replicate before plating, leading to a higher number of colonies that may share similar insertion profiles. This would mean that some of the additional colonies formed under longer recovery times are clones or near-clones of each other, representing the same or very similar insertion events.
- Saturation of Potential Sites: It's also possible that there is a kind of saturation effect where, despite more cells becoming transformants, the transposome tends to integrate into a somewhat fixed repertoire of favored genomic sites. Thus, while more cells are transformed, the variety of unique genomic integration events does not proportionally increase.
* Conclusion Regarding Diversity: In summary, more colonies after a longer recovery time do not equate to a higher diversity of insertion site combinations. Instead, the diversity of insertion sites in terms of unique integration events remains consistent, indicating that more time allows for greater cell survival and colony formation, but not necessarily a greater diversity in the genetic outcomes of those colonies.
This conclusion can be valuable in experimental design and interpretation in genetics, particularly when using transposon mutagenesis to explore gene function, as it highlights the importance of considering both the number of transformants and the actual diversity of genetic alterations achieved through the process.
Selecting mutants in liquid broth may be a viable alternative to construct a complex mutant library
- Constructing the TraDIS mutant library is a laborious task.
- To achieve the desired complexity of the library, it is necessary to prepare, plate, and scrape (/skreɪp/) hundreds of agar plates to collect hundreds of thousands of colonies.
- A potential alternative to this method is to select the bacteria in a pooled fashion using liquid broth with a selection agent.
- Previously, Fels and colleagues developed a variant of the Tn-seq approach called Transposon Liquid Enrichment Sequencing (TnLE-seq) and demonstrated that a liquid broth can be successfully used to build a complex mutant strain library of Desulfovibrio vulgaris Hildenborough9.
- However, only a handful of studies have adopted the strategy10,11, despite its potential to save time, labor and cost.
- We sought to examine whether the liquid broth approach could be adopted for constructing complex mutant libraries after electroporation of Tn5 mini-transposons.
- We electroporated our E. coli cohort with the Tn5 transposome carrying the KAN2 selection gene, and after 1 h of recovery, selected the resulting transformants either: (1) by plating them onto LB-kanamycin plates and collecting colonies after overnight incubation; or (2) by incubating them in a liquid broth with kanamycin and later collecting as the culture reached early-, mid-, or late log growth phase.
- All experiments were done in duplicate.
- We isolated DNA from each condition and determined the number of unique mutants via sequencing after subsampling reads to 5 million for each strain
and condition (Table 1).
- For two of the three test strains (AB_00116 and MS 69-1), we found that the number of unique mutants detected were comparable between the two selection methods; whereas, for one of the test strains (D43HC1), we observed on average 15% reduction in the number of mutants when liquid broth was used for selection. For all strains, the growth phase of the liquid cultures at the time of harvest did not affect the number of unique mutants (0 = 0.44).
- NOTE that the Tn5 mini-transposon corresponds to the "KAN2 transposon DNA" part in the information you provided. In the assembly of the Tn5 transposome, the Tn5 mini-transposon would be the DNA component that pairs with the Tn5 transposase (the protein) to form the Tn5 transposome, which is a protein-DNA complex.
- NOTE that in the context provided, "unique mutants" refers to individual bacterial cells in which the Tn5 mini-transposons have inserted into different genomic locations, resulting in genetic variations that are distinct from each other. Each unique mutant represents a different insertion site of the transposon within the bacterial genome. This variation is crucial for constructing a complex mutant library because it allows for a wide exploration of gene functions and interactions within the organism.
Nextera‐TruSeq hybrid library design enables a simple PCR approach to enrich transposon‐DNA junctions
Discussion
- Transposon insertion sequencing is a powerful high-throughput method of identifying essential bacterial genes in various growth conditions.
- Yet, there are several key technical hurdles that one has to overcome to successfully conduct the assay: an efficient construction of a complex mutant library, and a robust detection of the resulting transposon-DNA junctions using deep sequencing.
- In this study, we have identified a number of electroporation parameters that are readily adjustable that can enhance the number of transformants, and also described a simpler sequencing library workflow that is more cost-effective and flexible than previous approaches.
-
We believe that these technical improvements can make this powerful technique more accessible for a wider audience.
-
We found that more dilute concentration of the Tn5 transposome was more effective in generating higher CFUs, at least among our cohort of three E. coli strains we tested, as the optimal concentrations ranged between 10 and 20 times lower than the concentration frequently used in prior TraDIS studies (1 U).
- Poorer performance at higher transposome concentrations is likely due to the carryover of the Tn5 storage buffer into electroporation reactions, whose salt content may interfere with its efficiency.
- In support of this, reactions with higher transposome quantity had parallel decrease in the time constant, which is often a good indicator of electroporation efficiency.
-
On the other hand, overly diluted transposome solutions result in a low overall yield of viable mutants, although these dilutions do not necessarily compromise the electroporation process itself as indicated by time constant.
-
The ability to use more diluted transposomes has several advantages.
- First, since the commercially available Tn5 transposase is an expensive reagent of the TraDIS assay, this reduces the overall cost of each electroporation
reaction by 10–20 fold.
- Second, because less amount of the enzyme is required for each round of electroporation, this allows researchers to optimize electroporation conditions directly using Tn5 transposomes, instead of using other molecules like plasmids as a proxy, whose optimized parameters may not be necessarily the same as those for Tn5 transposomes.
- Optimization of optical density (OD) and/or growth phase prior to electroporation is another well-documented factor influencing mutant yields (PMID: 35804085, PMCID: PMC3939052) that may vary between bacteria.
- As our experiments were specific to E. coli, we acknowledge that researchers applying these methods to other bacterial species might need to consider the OD/growth phase as a critical parameter.
-
More experiments are required to see if our findings are generalizable to other microbial species.
-
We also observed that the transposon concentration during transposome assembly has a striking impact on CFU recovery.
- A standard protocol (EZ-Tn5 Transposase manual) suggests the pairing of 200 ng of a transposon with 0.5 μM of Tn5 transposase (53.3 kDa) during assembly.
- We found that doubling the quantity of transposon to 400 ng enhanced CFUs by roughly fourfold, while halving the quantity to 100 ng reduced CFUs virtually to none.
- Our findings clearly show that using an insufficient amount of transposon during transposome assembly is detrimental to obtaining viable mutants, and therefore, a proper calculation of the molar ratio between the Tn5 transposase and the transposon is crucial.
- In our most successful condition with 400 ng of the KAN2 transposon (1221 bp), the molar ratio during assembly was roughly 15:1 Tn5 transposase to the transposon.
- Because a complete transposome requires 2 molecules of Tn5 for every molecule of transposon, 400 ng of the KAN2 transposon would allow us to form at maximum ~ 15% of complete transposomes in the assembly mixture.
- Therefore, it is theoretically possible that adding more quantities of transposons can yield higher CFUs; however, we did not test this in the current study.
- Similar to the Tn5-to-transposon ratio during assembly, our analysis also found that the cell density during electroporation reactions is another factor that can impact CFUs.
-
We found that concentrating a greater number of cells does not necessarily yield a higher number of viable mutants, and that there were strain-specific variations in the optimal densities.
-
When constructing the mutant libraries, CFUs are often used as a proxy for the number of unique mutants until sequencing is complete.
- While this is often a good proxy, existing TraDIS studies report a wide range of the CFU-to-unique mutant ratio (10–90%), and it is hard to draw conclusions about what parameters might be affecting this ratio.
- To address this, we investigated one potential factor influencing this relationship by varying the length of recovery time after electroporation.
- We reasoned that longer recovery time would result in higher CFUs but would also result in a lower proportion of unique mutants as longer incubation might facilitate expansion of clones.
- As expected, longer recovery time generated higher CFUs with an estimated doubling time of 0.5 h for our E. coli cohort.
-
However, contrary to what we expected, our results showed that the number of unique mutants did not decline in proportion; therefore, a longer recovery time may increase the overall yield of unique mutants perhaps by allowing more time for transposomes to mutate the genome.
-
Constructing the TraDIS mutant library is a laborious and time-consuming task.
- Inspired by the success of TnLE-seq, a variant of Tn-seq method that uses liquid broth as a selection medium to construct a complex mutant library, we explored the possibility of adopting this approach to TraDIS.
- Based on the side-by-side comparison of the number of unique mutants obtained from agar plates or liquid broth, our results suggest that liquid broth may be a viable option for selecting mutants for the TraDIS assay.
- Because of the pilot nature of our study, we intentionally kept the complexity of our mutant libraries low (~ 10,000 unique mutants per condition).
-
Future studies should address whether more complex libraries can be built using the liquid broth method.
In a typical transposon mutagenesis experiment, the terms "unique insertion sites" and "unique mutants" are often used interchangeably because each unique mutant is defined by a unique insertion of the transposon into the genome. Thus, if you have identified 10,000 unique mutants, this usually implies that you have also identified 10,000 unique insertion sites. Each mutant corresponds to a single, distinct insertion site where the transposon has integrated into the genome.
Here's how the terms align:
* Unique Mutants: Each represents a different bacterial cell or clone that carries the transposon inserted at a distinct location in the genome.
* The uniqueness of each mutant is determined by this unique insertion site.
* Unique Insertion Sites: These are the specific genomic coordinates where the transposon has landed. Each site is unique to a particular mutant.
In other words, the number of unique insertion sites should typically match the number of unique mutants in a well-controlled experiment, as each unique insertion site gives rise to a unique mutant. However, if there are methodological errors, such as cloning or sampling biases, or if multiple clones of the same insertion are picked up, the numbers might not perfectly align. But under ideal experimental and sequencing conditions, these two numbers should be equivalent.
-
Successful TraDIS assay also relies on efficient detection of transposon-DNA junctions via deep sequencing, and in this study, we introduced a simpler library design that does not involve the ligation of custom adaptors, custom sequencing primers during a sequencing run, or custom operation protocol of the sequencer such as the need for dark cycles.
- Despite its simplicity, our Nextera-TruSeq layout design generated a high proportion (~ 80%) of reads that correspond to transposon-DNA junctions. This is likely due to the ‘hybrid’ nature of the fragment layout because the same library preparation workflow failed if both ends of the fragments had TruSeq adaptors, as less than 0.5% of sequenced fragments corresponded to transposon-DNA junctions.
- The suboptimal performance of the TruSeq-TruSeq fragment layout can be attributed to the mechanics of the two-stage PCR process.
- Like previous approaches, the first round of PCR is designed to specifically amplify DNA fragments that contains transposon-DNA junctions; however, in the 2nd round of PCR, indexing primers are targeted to partial adaptor sequences that would also be present on all DNA fragments even if they do not contain junctions (Fig. 5).
- Consequently, depending on the relative proportion of target DNA and non-specific DNA fragments at the end of the first PCR, non-specific DNA can dominate the sequencing outputs.
-
The Nextera-TruSeq hybrid layout minimizes this type of non-specific amplification during index PCR because only target junction fragments would have the partial Nextera adapter on their 5’ end.
-
Our library design is also simpler in terms of indexing, as it uses the standard TruSeq and Nextera indexing primers that are compatible with other Illumina sequencing assays.
- Therefore, adapting the assay to a different transposon simply involves replacing the primers in the first PCR to target transposon-specific sequences, which is more cost-effective than synthesizing long all-in-one primers.
- Although we did not directly demonstrate this versatility in the current study, our hybrid library design should be compatible using other DNA library preparation kits, such as Illumina DNA Prep kit that integrates Nextera adaptors during tagmentation.
-
In this instance, one should set up the first round of PCR using a transposon-specific forward primer with the TruSeq i5 adaptor, and a reverse primer that targets the Nextera i7 adaptor to generate TruSeq-Nextera library fragments to minimize non-specific amplification.
-
TraDIS protocols still face several challenges that require improvement.
- Firstly, the efficiency and uniformity of transposon insertion across the genome needs enhancement to ensure comprehensive mutagenesis coverage.
- Even with strains that are amenable to genetic manipulation, the transformation efficiency using the transposome complex or plasmid vector, along with specific growth parameters and timing, can significantly limit mutant production and the accuracy of downstream analysis in TraDIS protocols.
- Secondly, data analysis pipelines must be optimized to identify and quantify insertional mutants accurately.
- Lastly, the development of more scalable library construction methods is necessary to facilitate the study of diverse organisms, enabling broader applications of TraDIS in functional genomics.